Growing up in Rajasthan, northwest India, Anil Kumar can’t remember a time when he wasn’t asking questions about how the world works. His natural curiosity turned academic when a gifted high school physics teacher introduced him to the mysteries that physicists explore, from the smallest particles to the composition and workings of the universe.
He was particularly intrigued by how quantum physics could explain phenomena like the behavior of systems composed of many interacting particles such as atoms: a complex puzzle known as the many-body problem. His path to becoming a theoretical physicist was set when he was able to solve a theoretical problem assigned by his physics teacher to determine the probable position of a freely moving particle in a three-dimensional box by calculating the pattern of waves the particle creates.
“That’s when I realized that science could be more than just about learning facts,” says Kumar. “I could combine my love of problem solving with hands-on research to explore and understand the universe by testing theories and conducting experiments.”
After obtaining a Bachelor of Science degree from a college affiliated with the University of Rajasthan in 2013, and then a Master of Science in Physics from the University of Rajasthan, in 2015, he went on to earn a Ph.D. in theoretical nuclear physics at the Indian Institute of Technology Roorkee (IITR), in the northern state of Uttarakhand, in 2021. He then spent the next 18 months teaching physics as an assistant professor at JECRC University, Jaipur, as well as conducting research at IITR. During this time, he heard about a new research program established in 2020 by Japan’s Ministry of Education, Culture, Sports, Science and Technology (MEXT) called the Program for Promoting Research on the Supercomputer Fugaku.
Supercomputer Fugaku
Fugaku, named after Japan’s iconic Mount Fuji, is installed in the RIKEN Center for Computational Science in Kobe and is Japan’s flagship supercomputer and one of the world’s most powerful supercomputers. It is designed to handle diverse and complex computational tasks. Hence the MEXT initiative is broad and aims to address such science and social challenges as disaster prevention, earthquake prediction, and environmental challenges. Additionally, it aims to boost Japan’s industrial competitiveness in high-performance computing, with applications in drug discovery, advanced manufacturing, and exploring fundamental physics and astrophysics, for instance.
Kumar applied to take part in the program and was invited to join a project focusing on conducting simulations in basic science to prepare for the coming quantum era, one of nineteen projects comprising the MEXT scheme. The project is led by Shoji Hashimoto at the High Energy Accelerator Research Organization known as KEK, in Tsukuba, Japan’s Science City. The project covers several research themes, and in August 2023, Kumar was assigned to begin post-doctoral research at the Center for Computational Science (CCS) under Professor Noritaka Shimizu at the University of Tsukuba.
“My long-term research goal is to gain a comprehensive understanding of the nuclear structure and nuclear astrophysics and then work in an academic research lab or comparable industry-based lab in India,” says Kumar. “The University of Tsukuba is renowned for its research programs in nuclear physics and computational sciences. So, I believe conducting post-doctoral research at the CCS will provide me with the finest training and help me achieve my goal.”
Nuclear Shell Model
Kumar’s work at CCS is concerned with the study of nuclear structure through quantum many-body computation, which involves heavy use of the nuclear shell model.
The nuclear shell model is a kind of mathematical map or framework that describes how protons and neutrons (collectively known as nucleons), are arranged or spread out across different energy levels, or “shells,” within an atomic nucleus (Fig. 1). These shells determine the energy levels of the nucleons and understanding them helps elucidate the behavior of the nucleus.
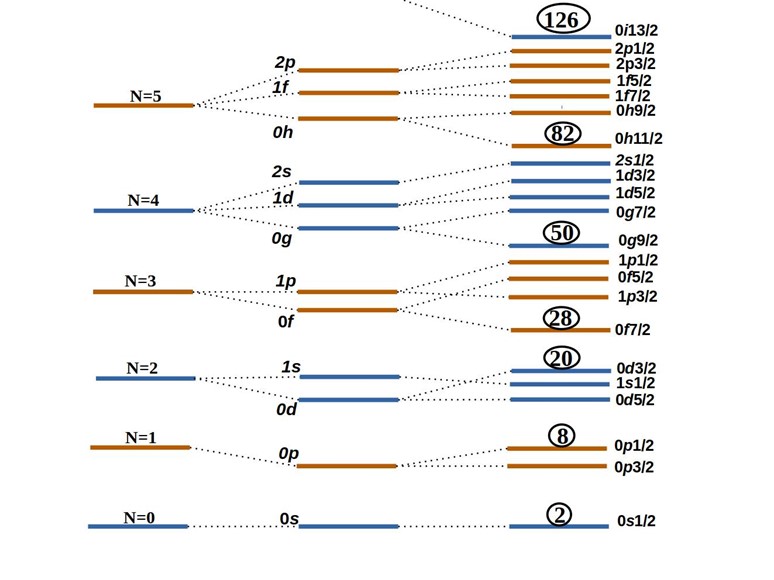
Fig. 1: The left column represents the principal quantum shell numbers, the middle column lists the orbital designations for each energy level, and the right column shows the split orbitals after spin-orbit coupling accounting. The magic numbers of nucleons are indicated within the circles.
“The beauty of the shell model lies in its ability to explain the magic numbers of protons and neutrons,” says Kumar, referring to specific numbers of nucleons that result in particularly stable nuclear configurations. “By using the shell model to study nuclear structures, we can gain a better understanding of phenomena such as nuclear spin, magnetic moments, and the overall stability of different isotopes.”
However, working with the shell model is computationally intensive as it involves calculating complex interactions between many particles in a nucleus. This approach often requires constructing large matrices that expand exponentially as the number of nucleons increases. Furthermore, researchers must account for various quantum effects, not the least the Pauli Exclusion Principle, which states that no two protons or neutrons can occupy the same quantum state simultaneously.
“But the project I joined at CCS has access to Fugaku, Japan’s most powerful supercomputer,” says Kumar. In addition, he notes that the university is equipped with three supercomputers: Pegasus, Wisteria, and Cygnus, which he can access to carry out additional complex and detailed calculations that employ the nuclear shell model.
Beta Decay
It is well established that elements heavier than iron are created when lighter atomic nuclei capture neutrons in a process called nucleosynthesis. This can occur at different rates. During beta decay, a form of radioactive decay, a neutron is converted to a proton (or vice versa), with the emission of an electron and a neutrino. When neutron capture occurs at a slower rate than beta decay, it is known as the slow-neutron capture process (s-process). In the s-process, heavier elements are synthesized along the lines of stability. Conversely, when neutron capture is faster than beta decay, it is called the rapid-neutron capture process (r-process), which produces a wide variety of neutron-rich isotopes, many of which are unstable.
There are two possible types of transitions in single beta decay: Gamow-Teller (GT) transitions and first-forbidden (FF) transitions (named for its rare occurrence). Kumar and his colleagues have investigated how these two types of beta decay influenced the half-lives of the nuclei of atoms with 126 or 125 neutrons, irrespective of the number of protons. Nuclei with 126 neutrons are of particular interest because 126 is a “magic number,” representing a highly stable nuclear (as was noted earlier).
Specifically, the researchers calculated the half-lives of these nuclei as the number of protons increased. When both GT and FF transitions were included in the calculations, the results matched available experimental data better than that produced by existing theoretical models, and better than their own model when it included only the more common GT transitions (Fig. 2).
The researchers also explored how the two types of transitions contribute to the half-life process. They found that in nuclei with 70 or more protons, the less common first forbidden transitions significantly impact the decay rates of atomic nuclei with 126 or 125 neutrons. In contrast, nuclei with fewer than 70 protons, the more common Gamow-Teller transitions dominate the decay process (Fig 3.)
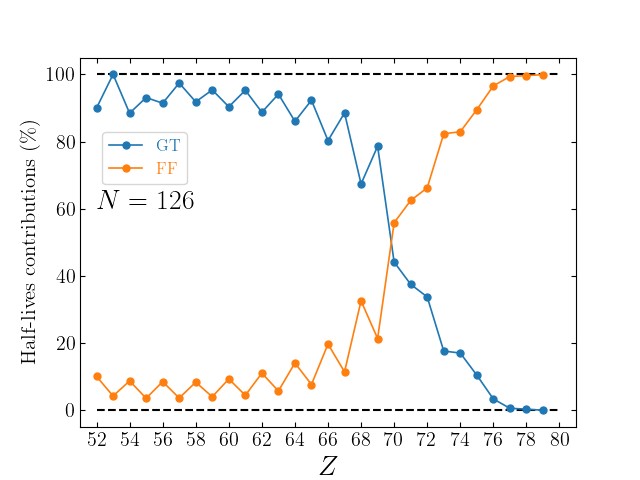
Fig. 3: Contribution of FF transitions and GT transitions in half-lives of atomic nuclei with 126 neutrons.
Additionally, the researchers also investigated another measurable quantity, the beta-delayed neutron emission probability, which refers to the likelihood of a nucleus emitting a neutron after beta decay. Figure 4 shows calculated probabilities for isotones with neutron number N = 126 and proton numbers from 52 to 79. The results predict higher probabilities for odd-even nuclei (those with an odd number of protons and an even number of neutrons, or vice versa) compared to established predictive models like KTUY and FRDM models used by nuclear physicists. This decay process is crucial for heavy element formation in neutron-rich environments. Understanding these probabilities aids in predicting the behavior of neutron-rich nuclei during astrophysical events such as supernovae and neutron star mergers.
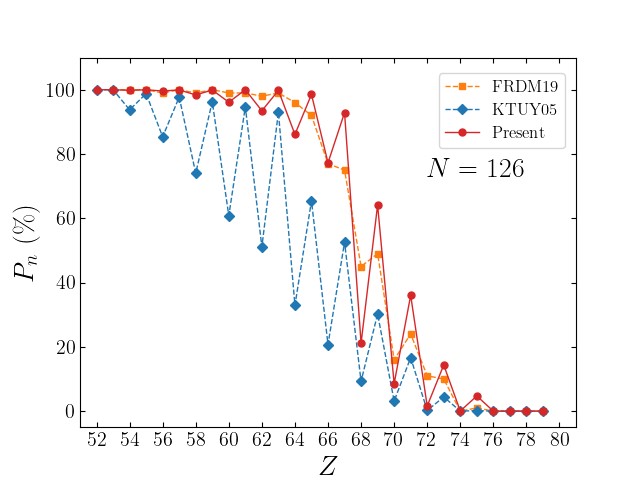
Fig. 4 Theoretical beta-delayed neutron emission probabilities of N = 126 isotones with varying proton numbers from Z = 52 to Z = 78.
Importance of Prediction
To predict how a nucleus will change, the researchers use the shell model to derive a complex mathematical formula called a nuclear matrix element (NME), which is based on the arrangement and behavior of particles in the nucleus.
On a practical level, this ability to predict changes in nuclear processes is important for several reasons. Beta decay significantly contributes to formation of elements in the universe, especially during events like a supernova. Therefore, understanding and predicting changes in nuclear decay enhances our knowledge of the universe’s evolution. In nuclear energy generation, controlling and predicting beta decay can improve its efficiency and safety. And in medicine, beta decay is also essential, playing an important role in medical applications such as in imaging and cancer treatment.
Going forward, Kumar and his colleagues will investigate how their predictions for beta decay affect the abundance of decay rates of heavy elements in space to deepen understanding of how heavy elements in the universe are created.
Research in Japan
Kumar says that working in Japan as a researcher differs from his prior experience in two significant ways. “The availability of advanced computational resources such as the Fugaku supercomputer provides me with unparalleled computing power for conducting large-scale simulations and complex calculations. Previously, access to high-performance computing resources was limited.”
The second difference is the strong emphasis put on collaboration and interdisciplinary research. “The environment here is quite different from my earlier experiences, where research was often specialized and confined,” says Kumar. “By contrast, members of the Nuclear Theory Group at Tsukuba University are very friendly, and I can discuss any research-related issues with them. This creates a supportive and productive atmosphere for research.”